Scientific contact: Spencer Klein, 510-486-5470, [email protected]
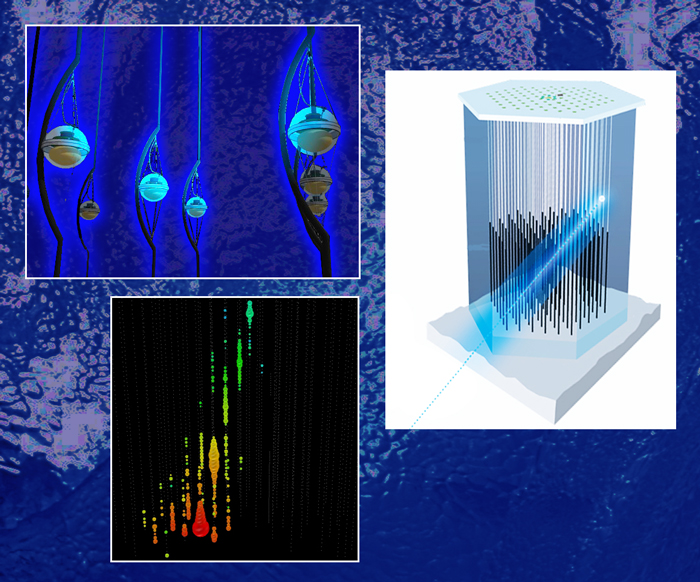
IceCube’s 5,160 digital optical modules are suspended from 86 strings reaching a mile and a half below the surface at the South Pole. Each sphere contains a photomultiplier tube and electronics to capture the faint flashes of muons speeding through the ice, their direction and energy – and thus that of the neutrinos that created them – tracked by multiple detections. At lower left is the processed signal of an energetic muon moving upward through the array, created by a neutrino that traveled all the way through the Earth.
The IceCube neutrino telescope encompasses a cubic kilometer of clear Antarctic ice under the South Pole, a volume seeded with an array of 5,160 sensitive digital optical modules (DOMs) that precisely track the direction and energy of speeding muons, massive cousins of the electron that are created when neutrinos collide with atoms in the ice. The IceCube Collaboration recently announced the results of an exhaustive search for high-energy neutrinos that would likely be produced if the violent extragalactic explosions known as gamma-ray bursts (GRBs) are the source of ultra-high-energy cosmic rays.
“According to a leading model, we would have expected to see 8.4 events corresponding to GRB production of neutrinos in the IceCube data used for this search,” says Spencer Klein of the U.S. Department of Energy’s Lawrence Berkeley National Laboratory (Berkeley Lab), who is a long-time member of the IceCube Collaboration. “We didn’t see any, which indicates that GRBs are not the source of ultra-high-energy cosmic rays.”
“This result represents a coming-of-age of neutrino astronomy,” says Nathan Whitehorn from the University of Wisconsin-Madison, who led the recent GRB research with Peter Redl of the University of Maryland. “IceCube, while still under construction, was able to rule out 15 years of predictions and has begun to challenge one of only two major possibilities for the origin of the highest-energy cosmic rays, namely gamma-ray bursts and active galactic nuclei.”
Redl says, “While not finding a neutrino signal originating from GRBs was disappointing, this is the first neutrino astronomy result that is able to strongly constrain extra-galactic astrophysics models, and therefore marks the beginning of an exciting new era of neutrino astronomy.”
The IceCube Collaboration’s report on the search appears in the April 19, 2012, issue of the journal Nature.
Blazing fireballs and nature’s accelerators
Cosmic rays are energetic particles from deep in outer space – predominately protons, the bare nuclei of hydrogen atoms, plus some heavier atomic nuclei. Most probably acquire their energy when naturally accelerated by exploding stars. A few rare cosmic rays pack an astonishing wallop, however, with energies prodigiously greater than the highest ever attained by human-made accelerators like CERN’s Large Hadron Collider. Their sources are a mystery.
“Nature is capable of accelerating elementary particles to macroscopic energies,” says Francis Halzen, IceCube’s principal investigator and a professor of physics at the University of Wisconsin-Madison. “There are basically only two ideas on how she does this: in gravitationally driven particle flows near the supermassive black holes at the centers of active galaxies, and in the collapse of stars to a black hole, seen by astronomers as gamma ray bursts.”
Klein, the deputy director of Berkeley Lab’s Nuclear Science Division (NSD, explains that in active galactic nuclei (AGNs) “the black holes suck in matter and eject enormous particle jets, perpendicular to the galactic disk, which could act as strong linear accelerators.” Of gamma-ray bursts he says, “Some GRBs are thought to be collapses of supermassive stars – hypernova – while others are thought to be collisions of black holes with other black holes or neutron stars. Both types produce brief but intense blasts of radiation.”
The massive fireballs move away from the explosion at nearly the speed of light, releasing most of their energy as gamma rays. The fireballs that give rise to this radiation might also accelerate particles to very high energies through a jet mechanism similar to that in AGNs, although compressed into a much smaller volume.
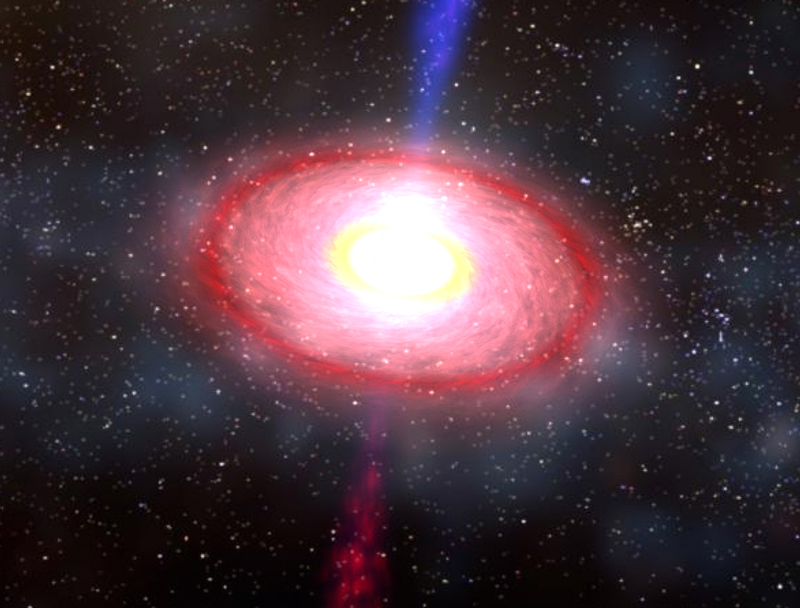
A fireball produced in a black-hole collision or by the collapse of a gigantic star can form jets in which protons and heavier nuclei are accelerated and shock waves produce a burst of gamma rays. The fireball model also predicts the creation of very high energy neutrinos, which ought to be detectable shortly after the gamma-ray burst becomes visible from Earth. (Image credit Dana Berry and NASA)
Accelerated protons in a GRB’s jets should interact with the intense gamma-ray background and strong magnetic fields to produce neutrinos with energies about five percent of the proton energy, together with much higher-energy neutrinos near the end of the acceleration process.
Neutrinos come in three different types that change and mix as they travel to Earth; the total flux can be estimated from the muon neutrinos that IceCube concentrates on. The muons these neutrinos create can travel up to 10 kilometers through the Antarctic ice. Thus many neutrino interactions occur outside the actual dimensions of the IceCube array but are nevertheless visible to IceCube’s detectors, effectively enlarging the telescope’s aperture.
“The way we search for GRB neutrinos is that we build a huge detector and then we just watch and wait,” says Klein. “When it comes to detecting neutrinos, size really does matter.”
IceCube watches with its over 5,000 DOMs, digital optical modules conceived, designed, and proven by Berkeley Lab physicists and engineers, which detect the faint light from each passing muon. Scientists can rely on their remarkable dependability to wait as long as necessary. Almost no failures occurred after the DOMs were installed; 98 percent are working perfectly and another one percent are usable. Now frozen in the ice, they will never be seen again.
IceCube records a million times more muon tracks moving downward through the ice than upward, mainly debris from direct cosmic-ray hits on the surface or secondary products of cosmic-ray collisions with Earth’s atmosphere. Muons moving upward, however, signal neutrinos that have passed all the way through Earth. When the telescope is searching for bright neutrino sources in the northern sky, the planet makes a marvelous filter.
Zeroing in on gamma-ray bursts
A network of satellites circles the globe and reports almost 700 GRBs each year, which readily stand out from the cosmic background. They’re timed, their positions are triangulated, and the data are distributed by an international group of researchers. Some blaze for less than two seconds and others for a few minutes. Neutrinos they produce should arrive at IceCube during the burst or close to it.
“IceCube’s precision timing and charge resolution, plus its large size, allow it to precisely determine where a neutrino comes from – often to within one degree,” says Lisa Gerhardt of Berkeley Lab, whose research has focused on detecting ultra-high-energy neutrino interactions. Indeed, a GRB neutrino should send a muon track through the ice with an angular resolution of about one degree with respect to the GRB’s position in the sky.
IceCube researchers sifted through data on 307 GRBs from two periods in 2008 and 2009 when IceCube was still under construction, looking for records of muon trails coincident in time and space with GRBs. (Forty strings, with 60 DOMs each, had been installed by 2008, and 59 strings by 2009. The finished IceCube has 86 strings.) The fireball model predicted that when the expected flux from all the samples had been summed, at least 8.4 related muon events would be found within 10 degrees of a GRB during the seconds or minutes when it was blazing brightly.
“Different calculations of the neutrino flux from GRBs are based on slightly different assumptions about how the neutrinos are produced and on uncertainties such as how fast the fireball is moving toward us,” says Klein. “Among the published predictions, the lowest estimate of neutrino production is about a quarter of what the fireball model predicts. That’s barely consistent with our zero observations.”
Says Halzen, “After observing gamma-ray bursts for two years, we have not detected the telltale neutrinos for cosmic ray acceleration.”
If it’s likely that GRBs aren’t up to the task of accelerating cosmic rays to ultra-high-energies, what are the options? Klein points to a salient fact about natural accelerators: a small, rapidly spinning object must accelerate particles very rapidly; this requires an extremely energy-dense environment, and there are many ways the particles could lose energy during the acceleration process.
“But remember the other popular model of ultra-high-energy cosmic rays, active galactic nuclei,” says Klein. “GRBs are small, but AGNs are big – great big accelerators that may be able to accelerate particles to very high energies without significant loss.”
Are AGNs the real source of the highest-energy cosmic rays? IceCube has looked for neutrinos from active galactic nuclei, but as yet the data sets are not sensitive enough to set significant limits. For now, IceCube has nothing to say on the subject – beyond the fact that the fireball model of GRBs can’t meet the specs.
###
“An absence of neutrinos associated with cosmic ray acceleration in gamma-ray bursts,” by R. Abbasi et al (the IceCube Collaboration), appears in the April 19, 2012, issue of Nature and is available online to subscribers at http://www.nature.com/nature/index.html. Collaboration members currently or formerly with Berkeley Lab include Keith Beattie, Kirill Filimonov, Lisa Gerhardt, Ariel Goldschmidt, Chang Hyon Ha, Spencer Klein, Howard Matis, Sandra Miarecki, David Nygren, Gerald Przybylski, Thorsten Stezelberger, and Robert Stokstad; Filimonov, Gerhardt, Ha, Klein, and Miarecki are also with the University of California at Berkeley.
The IceCube Collaboration includes over 260 researchers from 42 institutions in 11 countries and is supported by agencies and foundations in Belgium, Germany, Japan, and Sweden, with primary funding from the National Science Foundation and major support from the U.S. Department of Energy’s Office of Science. Visit the IceCube website at http://icecube.wisc.edu/, read the press release concerning this work at http://icecube.wisc.edu/news/view/52, and access a selection of images at http://icecube.wisc.edu/~norris/nature_press/.
At Berkeley Lab, DOE’s Office of Science supports participation in IceCube primarily through the National Energy Research Scientific Computing Center (NERSC). Visit http://www.nersc.gov/.
DOE’s Office of Science is the single largest supporter of basic research in the physical sciences in the United States, and is working to address some of the most pressing challenges of our time. For more information, please visit science.energy.gov.
Lawrence Berkeley National Laboratory addresses the world’s most urgent scientific challenges by advancing sustainable energy, protecting human health, creating new materials, and revealing the origin and fate of the universe. Founded in 1931, Berkeley Lab’s scientific expertise has been recognized with 13 Nobel prizes. The University of California manages Berkeley Lab for the U.S. Department of Energy’s Office of Science. For more, visit www.lbl.gov.