“A Search for a Permanent Electron Electric Dipole Moment,” awarded to David Kilcoyne, Benedict Feinberg, Harvey Gould, Juris Kalnins, Charles Munger, Jr., and Hiroshi Nishimura
An electron is a dimensionless point … isn’t it? Yet it has a magnetic dipole moment. Since an electron always has spin (in the quantum sense), it can be viewed classically as a spinning charge with north and south magnetic poles. Does spin also give the electron an electric dipole moment?
“If the electron were a line and not a point, you could imagine its charge distributed along the line,” says Benedict Feinberg of the Advanced Light Source (ALS). “So I like to say we’re trying to measure the length of the electron.”
No electric dipole moment has ever been found, and the Standard Model says it shouldn’t have one, at least not large enough to measure. But some important theories that go beyond the Standard Model – versions of supersymmetry, for example – predict a finite electric dipole moment for the electron.
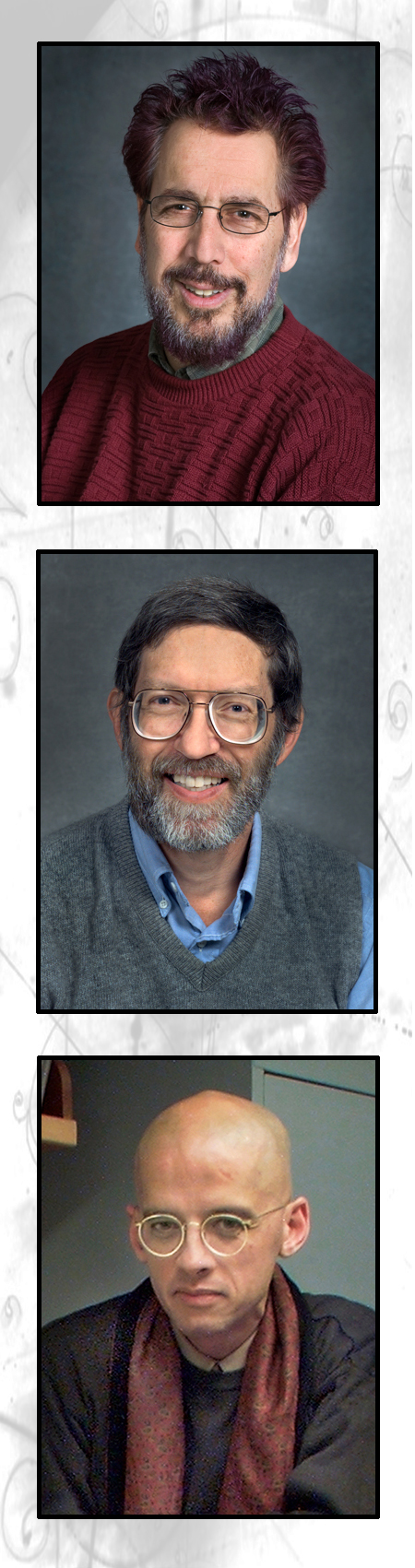
From top are Benedict Feinberg, Harvey Gould, and David Kilcoyne (not pictured are Juris Kalnins, Charles Munger, Jr., and Hiroshi Nishimura) (photos by Roy Kaltschmidt)
“Experimenters have already achieved sensitivities that suggest we ought to be able to see a dipole if it’s there, but they haven’t,” says the ALS’s Harvey Gould. “If a dipole moment doesn’t turn up when the experiments get more sensitive, it may cause trouble for some of these proposed extensions of the Standard Model.”
The collaboration of Feinberg, Gould, Juris Kalnins, David Kilcoyne, Charles Munger, Jr., and Hiroshi Nishimura (with more members being sought) will attempt the most sensitive measurement yet. The trick is to measure whether the energy of a neutral cesium atom changes according to whether its spin is aligned with an electric field or opposite to it.
“In the full-scale experiment, we’ll launch cesium atoms that are laser cooled to within a few millionths of a degree of absolute zero inside a vacuum chamber, and let them fall by gravity through the electric field,” Feinberg explains. “We know how they’re aligned with respect to the field, and by varying the field we can measure any tiny difference in energy.”
Any magnetic field, no matter how weak, can mask or destroy the effect. The interior of the experimental chamber must be isolated from even Earth’s magnetic field, through what Kilcoyne calls “a judicious choice of shielding.” The basic design is “nested cylinders of mu-metal” – a nickel-iron alloy that, like an invisibility cloak, effectively conducts magnetic field lines around the interior. Kilcoyne’s substantial experience in shielding design will help overcome a multitude of problems, most daunting the unavoidable gaps in the mu-metal shielding that admit wires, vacuum pumps, lasers, and so on.
The Discovery LDRD project is intended to learn whether it’s possible to build a sufficiently shielded space to perform the supersensitive experiment. Success will lead to the full-scale experiment, requiring a larger team of experts in numerous subsystems besides shielding, particle optics, and atomic physics, including laser trapping, cooling, and quantum state manipulation.
The results may lead to practical applications such as improved shielding for magnetic resonance imaging – and eventually to answers of fundamental questions in physical theory.
Read A Novel Route to Discovery, Part Four, describing an approach to an ingenious nanodevice for analyzing single-cell metabolomics.
Additional information
A Novel Route to Discovery, Part One
A Novel Route to Discovery, Part Two
Berkeley Lab’s Laboratory Directed Research and Development page