This video highlights magnet-making efforts to support the High-Luminosity Large Hadron Collider upgrade at CERN in Europe. Three U.S. Department of Energy national labs – Berkeley Lab, Fermilab, and Brookhaven Lab – are building superconducting magnets that can produce far stronger magnet fields than the magnets now in place at the LHC. This will enable more particle collisions and data to help us learn more about exotic particles and their properties. (Credit: Marilyn Sargent/Berkeley Lab)
This release was adapted from the original release prepared by Fermilab. View the original release.
In a multiyear effort involving three U.S. national laboratories, researchers have successfully built and tested a powerful new focusing magnet that represents a new use for niobium-tin, a superconducting material.
The eight-ton device – about as long as a semitruck trailer – set a record for the highest field strength ever recorded for an accelerator focusing magnet, and raises the standard for magnets operating in high-energy particle colliders.
The U.S. Department of Energy’s Fermi National Accelerator Laboratory, Brookhaven National Laboratory, and Lawrence Berkeley National Laboratory (Berkeley Lab) designed, built, and tested the new magnet.
It is one of 16 they will deliver for operation in the High-Luminosity Large Hadron Collider (HL-LHC) at CERN in Europe, which is an upgrade of the existing LHC – already the world’s most powerful particle accelerator. The 16 magnets, along with another eight produced by CERN, will focus beams of protons to a tiny spot as they approach collision inside two different particle detectors. The U.S.-based team will also deliver four spare magnets.
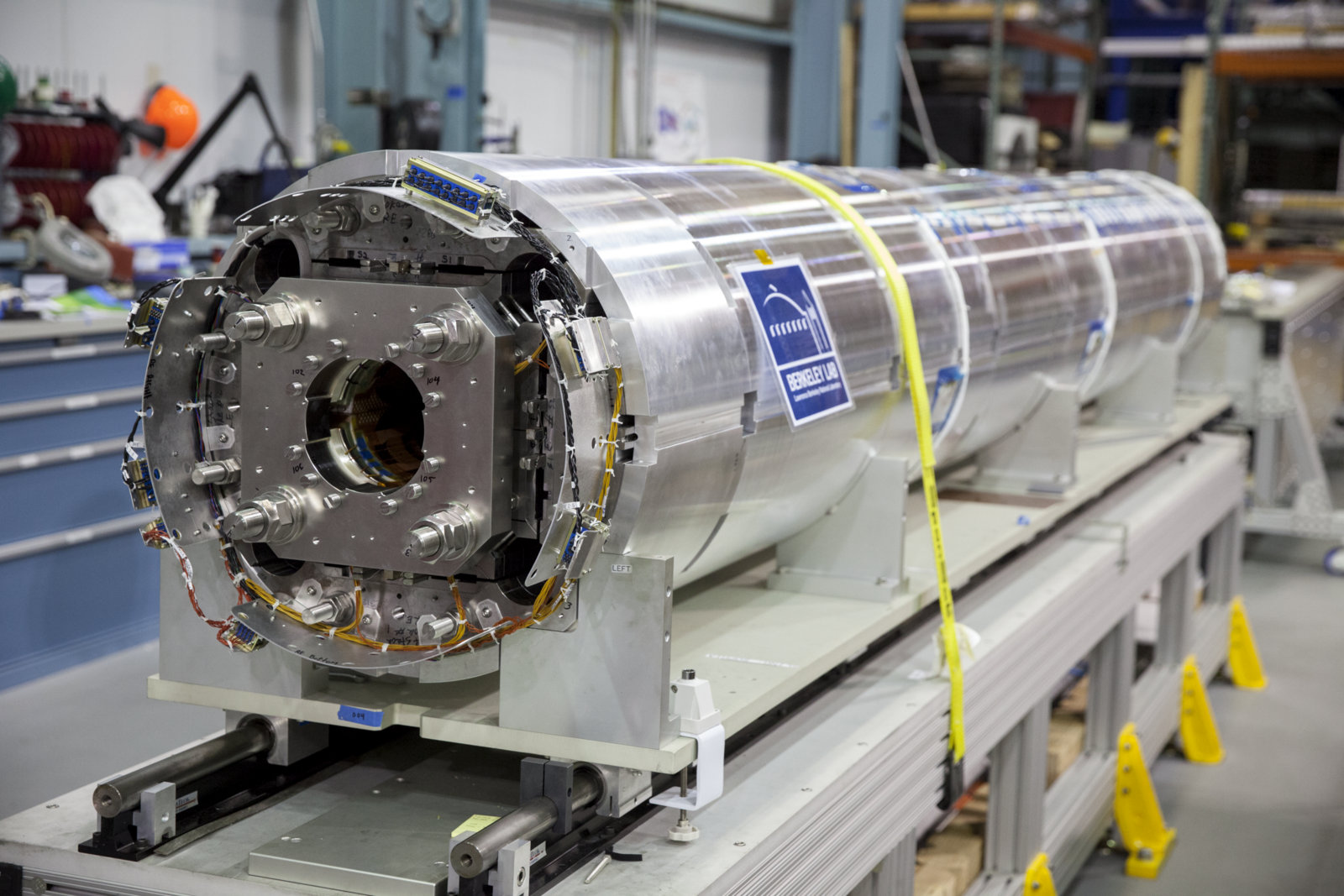
This magnet was built by teams at Berkeley Lab, Fermilab, and Brookhaven Lab for the High-Luminosity LHC upgrade project. (Credit: Marilyn Sargent/Berkeley Lab)
In all, the upgrade will require 130 new magnets of 11 different types, produced by more than a dozen international partners. The project will replace about three-quarters of a mile of equipment at the LHC.
Niobium-tin is the ingredient that sets these U.S.-produced magnets apart. It is a superconducting material that produces strong magnetic fields. These will be the first niobium-tin quadrupole magnets ever to operate in a particle accelerator.
Superconductivity is a state achieved by extremely cooling the magnets to a temperature hundreds of degrees below freezing. In this state, the magnets can pass electrical current with virtually no electrical resistance in order to maintain a tight focus of the particle beams.
The LHC is already the planet’s most powerful particle accelerator, and its high-luminosity successor will smash together beams of protons cruising around the 17-mile ring at close to the speed of light.
The High-Luminosity LHC will pack an additional punch: With its more intense beams, it will provide 10 times the collisions that are possible at the current LHC. With more collisions there are more opportunities to uncover new physics. The new focusing magnets will help it achieve that leap in luminosity delivered to the experiments.
Berkeley Lab’s work is focused on winding wires into thin cables, measuring and analyzing those cables to ensure they meet exacting requirements, testing the quality of the magnetic fields generated by the cable-formed magnet coils, assembling the magnets into support structures, and ensuring their proper alignment and uniform compactness.
Giorgio Apollinari, head of the three-lab U.S. LHC Accelerator Upgrade Project and a scientist at Fermilab, said, “We’ve demonstrated that this first quadrupole magnet behaves successfully and according to design, based on the multiyear development effort made possible by DOE investments in this new technology.”
Kathleen Amm, Brookhaven Lab’s representative for the Accelerator Upgrade Project, said, “It’s a very cutting-edge magnet, really on the edge of magnet technology.”
The new magnets, with higher field strength than those of the existing LHC, can bring particle beams to a tighter focus, resulting in more collisions that generate more data.
Focus, magnets, focus
In circular colliders, two beams of particles race around a ring in opposite directions. An instant before they reach the collision point, each beam passes through a series of magnets that focus the particle beams into a tiny spot, much like a magnifying glass focusing light rays to a point. Now packed as tightly as the magnets can get them, the beams collide.
Even with the new magnets, most of the particles won’t collide; they continue their paths around the ring until they get another collision opportunity at the next detector. But many particles do smash into each other. That number, and the scientific fruitfulness of that smash-up, depends on how dense the beam is. The more particles that are crowded together at the collision point, the greater the chance of collisions.
You get those tightly packed beams by sharpening the magnet’s focus. One way to do that is to widen the lens.
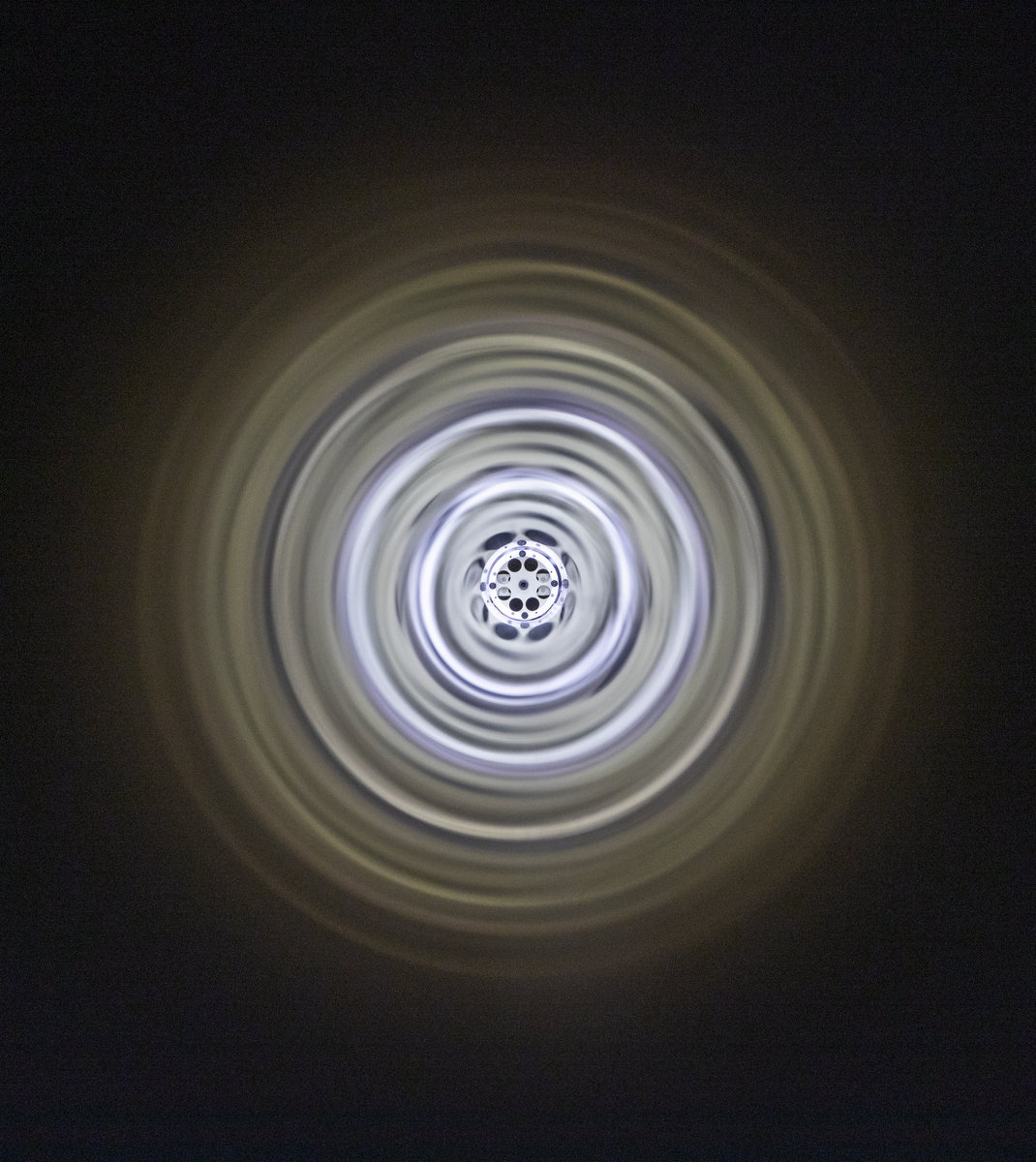
A magnet-testing instrument (center) is prepared for moving through the center of a magnet assembly at Berkeley Lab. (Credit: Marilyn Sargent/Berkeley Lab)
Consider the magnifying glass example: “If you try to focus the light from the sun using a magnifying glass at a small point, you want to have a more ‘powerful’ magnifying glass,” said Ian Pong, a Berkeley Lab scientist who oversees cable fabrication for the U.S. labs’ magnet effort. A larger lens has more light-gathering ability and stronger light-ray-bending power at its outer rim than a smaller lens.
In this analogy, the size of the lens is like a magnet’s aperture – the opening of the passageway the beam takes as it barrels through the magnet’s interior. If the beam is allowed to start wide before being focused, more particles will arrive at the intended focal point – the center of the particle detector. The U.S. focusing-magnet team widened the aperture to 150 millimeters, more than double the current LHC focusing magnet aperture of 70 millimeters.
But a wider aperture isn’t enough. There must be a strong magnetic field to actually focus the beam.
“The magnet has to squeeze the beam more powerfully than the LHC’s present magnets in order to create the luminosity needed for the HL-LHC,” Apollinari said.
To meet the demand, scientists designed and constructed a muscular focusing magnet. They calculated that, at the required aperture for the upgrade project, each focusing magnet would have to generate a field of between 11.4 and 12.4 teslas. This is up more than 50% from the 7.5-tesla field generated by the current niobium-titanium-based LHC magnets.
“So what do you do? You need to go to a different conductor,” Apollinari said.
Niobium-tin for the win
Magnet experts have been experimenting with one of those conductors, niobium-tin, for decades. Electrical current coursing through a niobium-tin superconductor can generate magnetic fields of 12 teslas – about 250,000 times stronger than the Earth’s magnetic field at its surface – and beyond.
But niobium-tin calls for entirely different magnet-construction techniques than the ones used with niobium-titanium, because the heat treatment that makes niobium-tin superconductive also makes it brittle.
“Once they’re reacted, it becomes a beautiful superconductor that can carry a lot of current, but then it also becomes brittle,” Apollinari said.
“If you bend it too much, even a little bit, once it’s a reacted material, it sounds like corn flakes,” Amm said. “You actually hear it break.” Niobium-titanium was easier to work with because it is pliable.
Over the years, scientists and engineers have figured out how to produce a niobium-tin superconductor in a form that is useful. Guaranteeing that it would hold up as an HL-LHC focusing magnet was another challenge altogether.
State-of-the-art physics and engineering makes the magnets
To produce large, intricate magnets that meet the demands of a collider, the group of three U.S. labs teamed up – each with its own areas of expertise and specialized facilities – under Fermilab’s leadership.
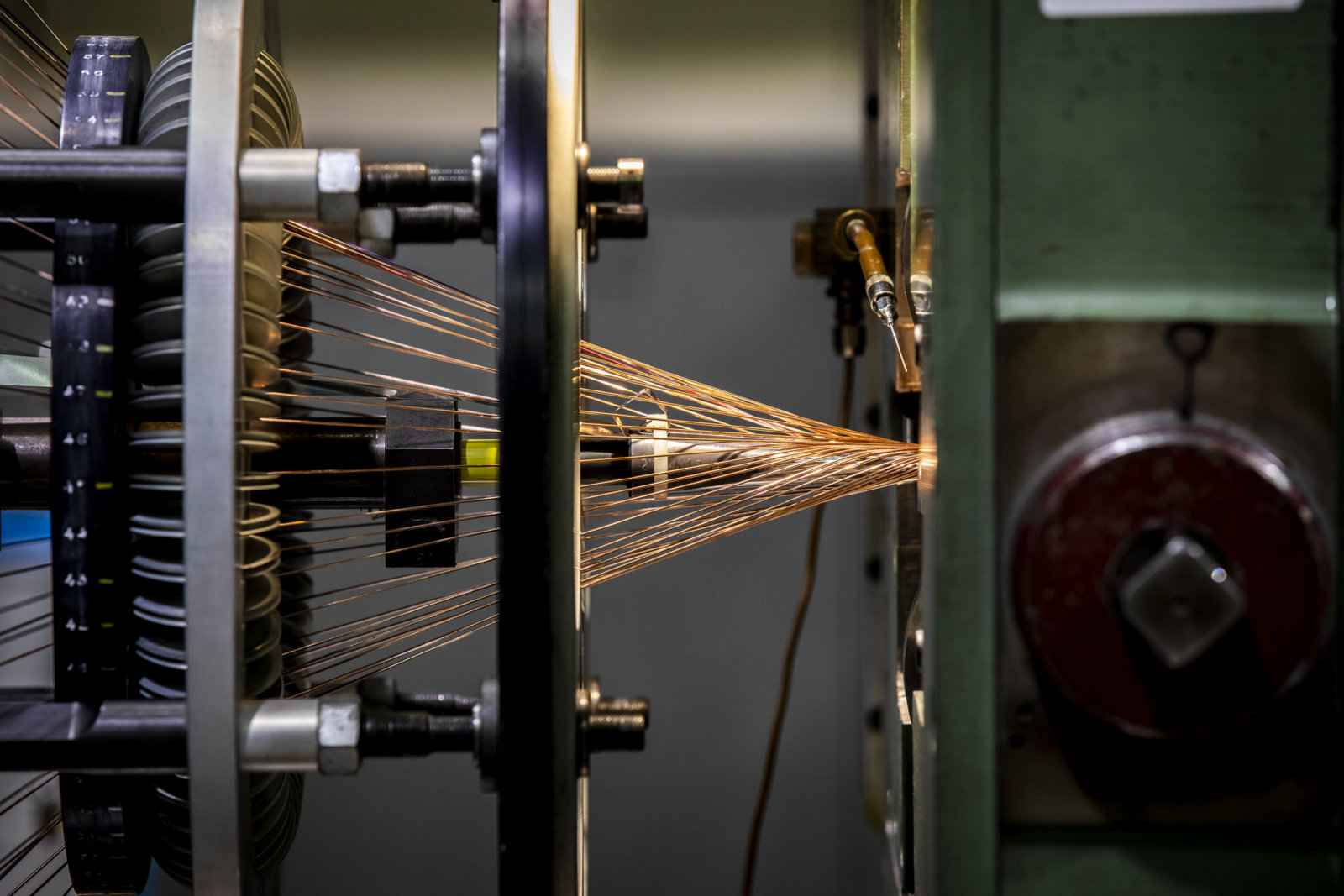
Spools of wire are wound into thinly pressed strips of cable at Berkeley Lab. The cable is wound to form niobium-tin magnet coils. (Credit: Marilyn Sargent/Berkeley Lab)
At Berkeley Lab, the magnet-making process begins by fabricating cable from 40 spools of wire – a copper matrix that contains niobium and tin. The machine-wound wires are rolled into thin, rectangular cables that must be defect-free and meet specifications within hundredths of a millimeter.
“Making these accelerator magnet cables is like directing 40 ballet dancers doing 5,000 pirouettes nonstop in a synchronized manner, where a single misstep would cost as much as crashing a few Tesla sportscars,” Pong said.
Scientists at Fermilab and Brookhaven wind these cables into coils, taking care to avoid excessively deforming them. Then comes a three-stage, weeklong heat treatment, causing a chemical reaction that makes the cables superconductive.
The magnet coils must be heated evenly, inside and out. “You have to control the temperature well. Otherwise the reaction will not give us the best performance,” Pong said. “It’s a bit like cooking. It’s not just to achieve the temperature in one part of the coil but in the entire coil, end to end, top to bottom – the whole thing.” Researchers make the coils strong and solid by embedding them in a resin.
It takes several months to yield a coil that is ready for service as one of a focusing magnet’s four poles. Together, the coils conduct the electric current that produces the magnetic field.
The magnet coils are assembled within an aluminum and steel support structure at Berkeley Lab to form a single magnet, and researchers test the magnetic fields produced by these coils and ensure that the support structure provides uniform pressure along the length of the coils.
Temporary water-pressurized metal bladders are used to provide tension to the support structure during assembly and alignment. The support structure is designed to withstand more than 20 million pounds of force during operation. Researchers use models and instruments to verify that the support structure can withstand the strength of the magnetic field.
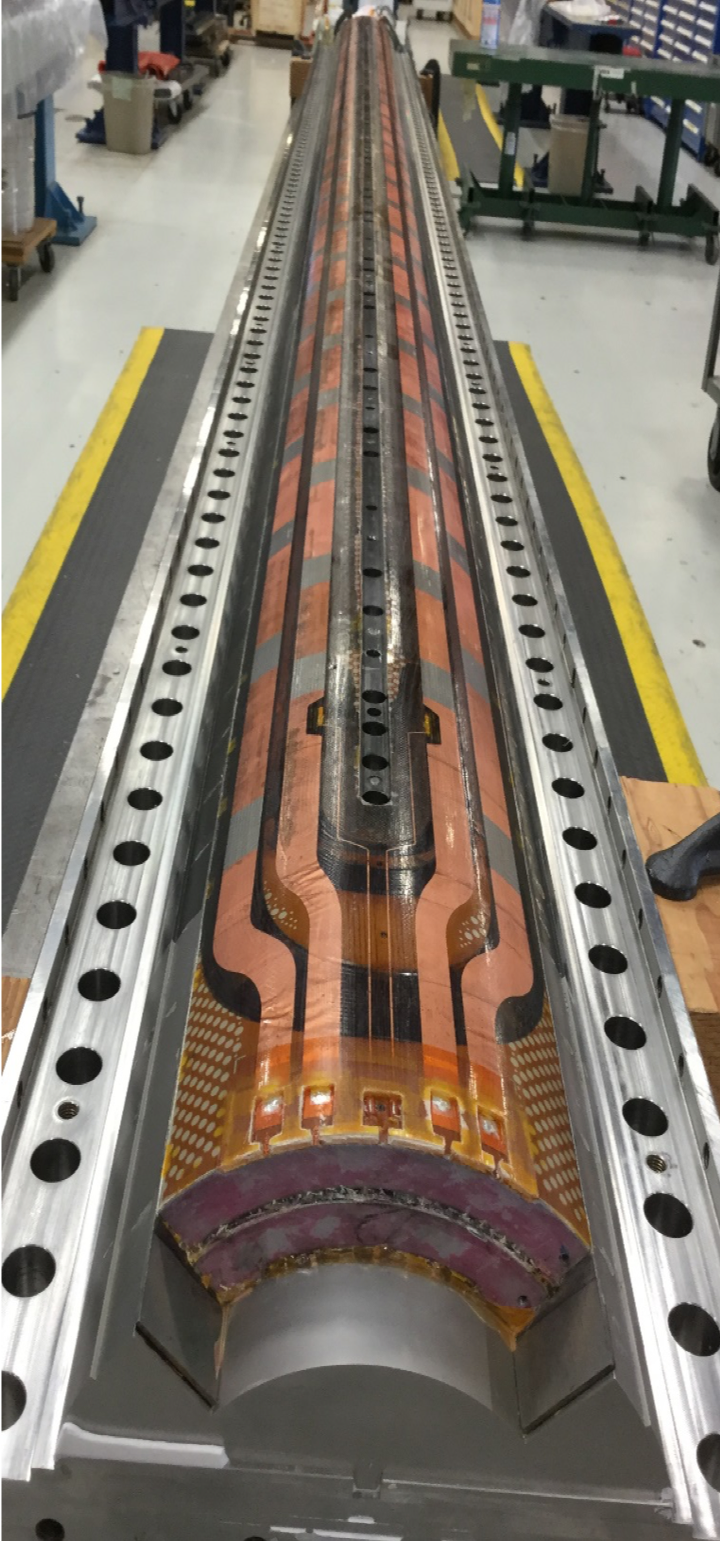
This completed niobium-tin magnet coil will generate a maximum magnetic field of 12 tesla, about 50 percent more than the niobium-titanium magnets currently in Cern’s LHC. (Credit Alfred Nobrega/Fermilab)
“Because these coils are very powerful when they are energized, there is a lot of force trying to push the magnet apart,” Pong said. “Even if the magnet is not deforming, at the conductor level there will be a strain, to which niobium-tin performance is very sensitive. The management of the stress is very, very important for these magnets.”
Alignment of the four coils within each magnet is also critical to performance. “You need very high field precision, so we have to have very high precision in how they align these to get good magnetic-field uniformity,” Amm said.
The full magnet is an eight-ton structure that’s about as long as a semitruck trailer. The magnets are shipped to Brookhaven for testing, then to Fermilab for installation in their cryogenic containers, followed by another round of testing before shipment to CERN.
“This will be the first use of niobium-tin in focusing accelerator magnets, so it will be pretty exciting to see such a complex and sophisticated technology get implemented into a real machine,” Amm said. “These magnets are currently the highest-field focusing magnets in accelerators as they exist today.”
Pong added, “Finally we are coming to it, and we really want to make sure it is a lasting success.”
The many moving parts of an accelerator collaboration
Ensuring lasting success has as much to do with the operational choreography as it does with the exquisite engineering. Conducting logistics that span years and a continent requires painstaking coordination.
“For example, transportation communication: We have to make sure that things are well protected,” Pong said. “Otherwise these expensive items can be damaged, so we have to foresee issues and prevent them.”
Amm, Apollinari, and Pong acknowledge that the three-lab team has met the challenges capably, operating as a well-oiled machine.
“The technologies developed at Fermilab, Brookhaven, and Berkeley helped make the original LHC a success. And now again, these technologies out of the U.S. are really helping CERN be successful,” Amm said. “It’s a dream team, and it’s an honor to be a part of it.”
The team’s achievements are made possible by many years of R&D. The U.S.-based Accelerator Upgrade Project for the HL-LHC, of which the focusing-magnet project is one aspect, began in 2016. It grew out of a 2003 predecessor, the U.S. LHC Accelerator Research Program (LARP), that developed LHC-related accelerator technology. And these efforts drew upon past experience in pushing the frontiers of magnet and materials performance for a variety of applications.
From now until about 2025, the U.S. labs will continue to build the LHC magnets, from fine strands of niobium-tin to the hulking finished products. In 2022 they plan to begin delivering the magnets to CERN. Installation is planned in the following three years.
“People say that ‘touchdown’ is a very beautiful way to describe the landing of an airplane, because you have a huge metal object weighing hundreds of tons, descending from the sky, touching a concrete runway very gently,” Pong said. “Our magnets are massive superconducting devices, focusing tiny, invisible particle beams that are flying close to the speed of light through the bore. It’s quite magical.”
The magic starts in 2027, when the High-Luminosity LHC comes online.
The work, Apollinari said, is “a generational passing of the baton.”
“The upgrade project exemplifies what can be achieved through co-operation among the laboratories,” said Associate Laboratory Director James Symons. “The U.S. contribution to the luminosity upgrade has been made possible by the very successful LARP collaboration, which developed the design concept over the past decade.”
This work is supported by the DOE Office of Science.
More:
- View the original Fermilab release.
- CERN Ceremony Marks Groundbreaking for Accelerator Upgrade Involving U.S. National Labs
- Successful Test of New U.S. Magnet Puts Large Hadron Collider on Track for Major Upgrade
- An Advance in Superconducting Magnet Technology Opens the Door for More Powerful Colliders
- World Record Magnet 300,000 Times Strength of Earth’s Magnetic Field
# # #
Founded in 1931 on the belief that the biggest scientific challenges are best addressed by teams, Lawrence Berkeley National Laboratory and its scientists have been recognized with 13 Nobel Prizes. Today, Berkeley Lab researchers develop sustainable energy and environmental solutions, create useful new materials, advance the frontiers of computing, and probe the mysteries of life, matter, and the universe. Scientists from around the world rely on the Lab’s facilities for their own discovery science. Berkeley Lab is a multiprogram national laboratory, managed by the University of California for the U.S. Department of Energy’s Office of Science.
DOE’s Office of Science is the single largest supporter of basic research in the physical sciences in the United States, and is working to address some of the most pressing challenges of our time. For more information, please visit energy.gov/science.