
The successive interaction of a high-power laser pulse (red and blue) with a plasma mirror (not shown) and a secondary target (translucent light grey) could create the conditions to probe Strong Field Quantum Electrodynamics effects that are far beyond current experimental capabilities. (Credit: Luca Fedeli/CEA).
-By William Schulz
A newly published theoretical and computer modeling study suggests that the world’s most powerful lasers might finally crack the elusive physics behind some of the most extreme phenomena in the universe – gamma ray bursts, pulsar magnetospheres, and more.
The international research team behind the study includes researchers from Lawrence Berkeley National Laboratory (Berkeley Lab) and France’s Alternative Energies and Atomic Energy Commission (CEA-LIDYL). They report their findings in the prestigious journal Physical Review Letters.
The research team was led by CEA’s Henri Vincenti, who proposed the main physical concept. Jean-Luc Vay and Andrew Myers, of Berkeley Lab’s Accelerator Technology and Applied Physics (ATAP) Division and Computational Research Division, respectively, led development of the simulation code used for the research. (Vincenti previously worked at Berkeley Lab as a Marie Curie Research Fellow and remains an ATAP affiliate and frequent collaborator.) The theoretical and numerical work was led by Luca Fedeli from Vincenti’s team at CEA.
The team’s modeling study shows that petawatt (PW)-class lasers – juiced to even higher intensities via light-matter interactions – might provide a key to unlock the mysteries of the strong-field (SF) regime of quantum electrodynamics (QED). A petawatt is 1 times ten to the fifteenth power (that is, followed by 15 zeroes), or a quadrillion watts. The output of today’s most powerful lasers is measured in petawatts.
“This is a powerful demonstration of how advanced simulation of complex systems can enable new paths for discovery science by integrating multiple physics processes – in this case, the laser interaction with a target and subsequent production of particles in a second target,” said ATAP Division Director Cameron Geddes.
Lasers probe some of nature’s most jealously guarded secrets
While QED is a cornerstone of modern physics that has withstood the rigor of experiment over many decades, probing SF-QED requires electromagnetic fields of an intensity many orders of magnitude beyond those normally available on Earth.
Researchers have tried side routes to SF-QED, such as using powerful particle beams from accelerators to observe particle interactions with the strong fields that are naturally present in some aligned crystals.
For a more direct approach, the highest electromagnetic fields available in a laboratory are delivered by PW-class lasers. A 10-PW laser (the world’s most powerful at this time), focused down to a few microns, can reach intensities close to 1023 watts per square centimeter. The associated electric field values can be as high as 1014 volts per meter. Yet studying SF-QED requires even higher field amplitudes than that – orders of magnitude beyond what can be achieved with those lasers.
To break this barrier, researchers have planned to call on powerful electron beams, accessible at large accelerator or laser facilities. When a high-power laser pulse collides with a relativistic electron beam, the laser field amplitude seen by electrons in their rest frame can be increased by orders of magnitude, giving access to new SF-QED regimes.
Though such methods are challenging experimentally, as they call for the synchronization in space and time of a high-power laser pulse and a relativistic electron beam at femtosecond and micron scales, a few such experiments have been successfully conducted, and several more are planned around the world at PW-class laser facilities.
Using a moving, curved plasma mirror for a direct look
The research team proposed a complementary method: a compact scheme that can directly boost the intensity of existing high-power laser beams. It is based on a well-known concept of light intensification and on their theoretical and computer modeling studies.
The scheme consists of boosting the intensity of a PW laser pulse with a relativistic plasma mirror. Such a mirror can be formed when an ultrahigh intensity laser beam hits an optically polished solid target. Due to the high laser amplitude, the solid target is fully ionized, forming a dense plasma that reflects the incident light. At the same time the reflecting surface is actually moved by the intense laser field. As a result of that motion, part of the reflected laser pulse is temporally compressed and converted to a shorter wavelength by the Doppler effect.
Radiation pressure from the laser gives this plasma mirror a natural curvature. This focuses the Doppler-boosted beam to much smaller spots, which can lead to extreme intensity gains – more than three orders of magnitude – where the Doppler-boosted laser beam is focused. The simulations indicate that a secondary target at this focus would give clear SF-QED signatures in actual experiments.
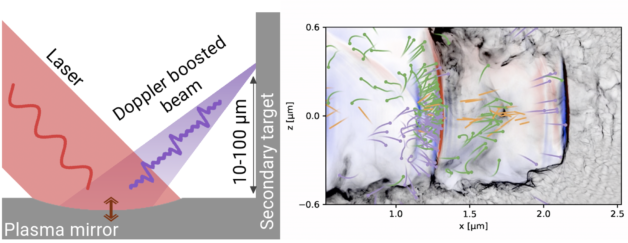
Left: In the proposed scheme for probing SF-QED with present-day or near-future lasers, a plasma mirror shaped by radiation pressure converts an intense laser pulse (red) into Doppler-boosted harmonics (purple) and focuses them on a secondary target, reaching extreme intensities. The dimensions involved are tens to hundreds of microns (millionths of a meter); the diameter of a human hair is a few to several tens of microns. (Credit: Luca Fedeli/CEA)
Right: Berkeley Lab’s key contribution was leading the development of the simulation code used for the research. In this simulation image, the intense Doppler-boosted light pulses (red and blue) plow through the solid target (gray), generating high-energy photons (orange) that decay into pairs of electrons (green) and positrons (purple) after further interaction with the incoming light pulses. The electrons and positrons are separated due to the strong laser field. Only photons that have not yet decayed into pairs are shown. (Credit: Luca Fedeli/CEA)
Berkeley Lab integral to international team-science effort
The study drew upon Berkeley Lab’s diverse scientific resources, including its WarpX simulation code, which was developed for modeling advanced particle accelerators under the auspices of the U.S. Department of Energy’s Exascale Computing Project. The novel capabilities of WarpX allowed the modeling of the intensity boost and the interaction of the boosted pulse with the target. All previous simulation studies had only been able to explore proof-of-principle configurations.
Experimental verification of the research team’s methodology for probing SF-QED might come from the Berkeley Lab Laser Accelerator (BELLA), a petawatt-class laser with a repetition rate, unprecedented at that power, of a pulse per second. Now under construction is a second beamline that might also contribute to experimental studies of SF-QED by Berkeley Lab researchers. A proposed new laser, kBELLA, could enable future high rate studies by bringing high intensity at a kilohertz repetition rate to the facility.
The discovery via WarpX of novel high-intensity laser-plasma interaction regimes could have benefits far beyond ideas for exploring SF-QED. These include the better understanding and design of plasma-based accelerators such as those being developed at BELLA. More compact and less expensive than conventional accelerators of similar energy, they could eventually be game-changers in applications that range from extending the reach of high-energy physics and of penetrating photon sources for precision imaging, to implanting ions in semiconductors, treating cancer, developing new pharmaceuticals, and more.
“It is gratifying to be able to contribute to the validation of new, potentially very impactful ideas via the use of our novel algorithms and codes,” Vay said of the Berkeley Lab team’s contributions to the study. “This is part of the beauty of collaborative team science.”
This work was supported by the French National Research Agency (ANR) T-ERC program, the European Union’s Horizon 2020 research and innovation program, and the Cross-Disciplinary Program on Numerical Simulation of CEA, the French Alternative Energies and Atomic Energy Commission. Berkeley Lab’s participation was supported by the Exascale Computing Project, a collaborative effort of DOE’s Office of Science and National Nuclear Security Administration. The simulations were run on the Summit supercomputer at Oak Ridge National Laboratory, using computer time awarded to “Plasma Mirrors ‘in Silico’: Extreme Intensity Light Sources and Compact Particle Accelerators” by the Innovative and Novel Computational Impact on Theory and Experiment (INCITE) program.
###
Founded in 1931 on the belief that the biggest scientific challenges are best addressed by teams, Lawrence Berkeley National Laboratory and its scientists have been recognized with 14 Nobel Prizes. Today, Berkeley Lab researchers develop sustainable energy and environmental solutions, create useful new materials, advance the frontiers of computing, and probe the mysteries of life, matter, and the universe. Scientists from around the world rely on the Lab’s facilities for their own discovery science. Berkeley Lab is a multiprogram national laboratory, managed by the University of California for the U.S. Department of Energy’s Office of Science.
DOE’s Office of Science is the single largest supporter of basic research in the physical sciences in the United States, and is working to address some of the most pressing challenges of our time. For more information, please visit science.energy.gov.